Answering Some Common Misconceptions About the Evolution of OrganismsAfter working as an assistant for an introductory level biology class and checking out message boards for some of the religious campus clubs I realize there are a lot of misconceptions circling around about evolution. This is no surprise as evolution is a subject that is often very poorly taught at a secondary school level. It is seen as being antagonistic to religion and so is barely touched upon. Another unfortunate thing is that at the college level, when people are confused about evolution, they are often too shy to ask because they fear they may be dismissed as being stupid or ignorant. Well, I’d like to clear things up without talking down, after all how can one know if one is never taught?
So, we’ll start off with some commonly misunderstood topics, along with the quote that pushed me to write about it.
1. Natural Selection
“How can new species just decide to be made? I don’t get how natural selection is supposed to make new animals.”
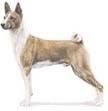
People have known about selection and evolution and used it to their advantage way before Darwin ever came on the scene. What in the world can I be speaking of? Why animal and plant breeding of course! Breeding is a fine example of evolution at work, but rather than being driven by natural selection it is driven by man’s selection. We breed animals with traits we find favorable until we have faster horses, better hunting hounds, juicier beef, or higher yield corn. Since it is well documented and we can even see the results of breeding within a lifetime, no one argues that this type of selection does not take place. But if we can do it, is it possible that nature can do the same thing if given the time?
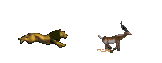
In the natural world, organisms that have less favorable traits are less likely to survive and reproduce. Just as breeders may put down a slow horse to eliminate the ill suited genes from their stock, the slower gazelle may be put down naturally by hungry lions on the savannah. Aside from predator/prey type relationships, organisms may be less fit to survive a number of other natural challenges such as weather or other environmental conditions, disease, food shortages, competition, or they simply may not be able to find a mate willing to help them pass on their heritable traits. All of these are considered forms of natural selection.
A funny thing to note about human and natural selection is that they sometimes collide in the most ironic ways. For instance, we’ve bred bananas to be big, high yield, and with minimal seed content. We haven’t given much thought to it’s susceptibility to diseases though and when natural selection hit banana crops with a case of Black Sigatoka, thousands died and the market got hurt pretty badly. Now it is a constant fungicidal battle to keep the banana killer at bay. Although bananas may meet our standards for being tasty and plump, they didn’t stand a chance against natural selection without us!

Cases like this happen all the time. Consider slow, dumb cattle being dragged down by wolves, crops being ravaged by disease, and let’s not forget the genetic abomination that is the chiwawa! It’s all a reminder of what man and nature can do to drive selection and create novel breeds or species.
2. Phylogenetic Trees
"Chickens are 94% similar in genetic makeup compared with humans, so does that mean that chickens evolved apes which evolved into us?"
This is probably the most common misconception people have toward evolution—that it is linear. Rather than seeing evolution as happening in a long line with all species ever existing somehow fitting in, let’s try to imagine it like an aged and expansive family tree, spanning millions of years and generations, with a mind-boggling amount of branches spreading every which way. When the possible relationships between organisms are represented this way, it is called a “
phylogenetic tree.” To get away from this idea that evolution is linear, take a look at your own family tree. You would not say that your second cousin came from your niece who came from you in a linear fashion, but you would say that you are related through a common ancestor. In the same way but much farther removed, chickens did not evolve into apes then humans, rather their ancestry all branch back to the trunk of the tree at some point. Think about your own family. You may look nothing like your second cousin, but you both shared a great grandparent. The farther removed a cousin is, the less they tend to look like you. This is similar to a phylogenetic tree, only different species are not just 3rd or 4th cousins, but one thousandth cousins! They’ve had hundreds or thousands of generations between each other, yet they share a common ancestor. In the case of mammals, the first mammal-like reptile is thought to be Therapsids, which were around in the Cretaceous period over 65 million years ago. Earlier still, the first bird-like reptile was thought to be Protoavis, which arose 225 million years ago. As you can see, we are not closely related to birds at all. There are millions of generations between us!
3. Homology
“I don’t understand why DNA is so important if animals are so different but so much of it is the same.”
Most of the DNA in animals is similar because what works tends to stick around. Why change a good plan? The DNA that is similar to all organisms is call homologous or conserved DNA. Just as you are likely to find more of your DNA is similar to your sister than your 2nd cousin, organisms that are more closely related tend to have more DNA in common as they have had less generations to diverge from the common ancestor. Often the DNA that is most retained over time are the portions of DNA which are used to make proteins that have similar functions in all animals. Really, a cat is not much different from a horse on the cellular level!
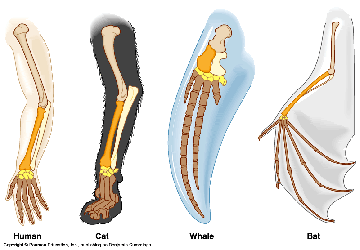
Many phylogenetic trees these days are constructed with the aid of genetic analysis, especially searching for homology and using rates of genetic change and mutation to develop a time frame. Finding homology in the morphology, or physical layout of organisms, is another common method of creating a phylogenetic tree. Many phylogenetic trees were constructed by examining bone structure combined with isotope dating. Isotope dating is when the rate of radioactive decay of isotopes (carbon, argon, lead, and other types of isotopes) is used to measure the age of the material it was found in. Most frequently, a combination of all methods are used to develop as accurate a phylogenetic tree as possible.
Check here to explore a gene based phylogenetic tree!:
genome.jgi-psf.org/tre_home.html4. Coexistence
“Evolutionists are trying to eliminate religion.”
Can science and religion get along? Of course. Many Christians have moved from the literal interpretation of the bible to a metaphorical view that allows them to accept an older age for the earth while still believing in God. I have heard some say, “a millennia is but a day in the eyes of God.” If you are a religious person and would like to maintain a literary view of the creation of the earth according to the bible, saying it is about 6,000 years old, then at least an appreciation of the modern application of science should be maintained. I have often heard it said that “God helps those who help themselves,” and science is a much needed tool for creating new technology that makes our world more efficient and medical advancements to increase the quality and length of life.
I encourage everyone to learn more about science, if for nothing else than at least to make better, more convincing arguments against what you feel is wrong.
Sources: http://www.akc.org
Images: lion and gazelle: http://wiki.gamer.free.fr/age-of-empires-1.html
Homology: http://www.anselm.edu/homepage/jpitocch/genbios/